Fluorination of organic molecules leads to unique physical and chemical properties, which are exploited in numerous commercial applications. Poly- and perfluorinated molecules tend to have low polarizability, which leads to increased volatility compared to non-fluorinated analogues. Thus, poly- and perfluoroalkyl substances (PFAS) are more likely to partition to the gas phase and thereby enter the atmosphere. The presence of PFAS in the atmosphere can have important implications. For example, long-range transport occurs much faster in the atmosphere than in other Earth spheres, enhancing the potential for global distribution and contamination.1 In addition, inhalation can be a source of human exposure to PFAS.2 In the atmosphere, PFAS can be present in both gaseous and particulate (solids or liquid suspended in gas) phases.3 Measurements of atmospheric PFAS in the context of outdoor and indoor air, as well as in laboratory experiments, are needed to better understand PFAS environmental sources, fate, and impacts.
Measurement of PFAS in atmospheric samples
Atmospheric deposition is a common method to assess the atmospheric loading of ionizable PFAS.4 This is a long-established method for collecting ionizable atmospheric species.5 It is advantageous because it provides an aqueous sample, which allows standard analytical methods for quantification of PFAS to be applied. An additional benefit of this approach is that PFAS present in atmospheric deposition samples are representative of the transfer from the atmosphere to the terrestrial/aquatic environment, which is of direct interest for ecosystem burden and global mobility research.
While these deposition samples are useful, the measurement of PFAS in the atmosphere in either gaseous or particulate form is important for species that do not readily deposit. Measurements of both phases come with a co-benefit of understanding the atmospheric chemical processing of PFAS. A challenge in atmospheric sampling is the separation of ultra-trace to trace target PFAS (sub-ppqv to pptv mixing ratios, or fg/m3 to ng/m3 mass loadings) from a high volume of atmospheric matrix. Based on this current range of anticipated atmospheric loadings, an effective separation is typically attempted in one of two ways by researchers: i) in situ measurement, where the separation and measurement are performed in real time, or ii) selective collection of target analytes paired with offline quantitative analysis.
Atmospheric chemists typically prefer the first approach, as it provides fast measurements that are on the same timescales as atmospheric processes, allowing for a more complete understanding of atmospheric fate and transport. One method that has measured atmospheric PFAS in situ uses iodide chemical ionization mass spectrometry.6,7 In this approach, an atmospheric sample is pulled through a pressure-controlled ion-molecule reaction chamber, and PFAS forms ionized adducts selectively with iodide. For example, the interaction of the 4:2 fluorotelomer alcohol to form a charged adduct that can be detected in real time by a mass spectrometer by this ionization scheme is:
C4F9CH2CH2OH + I- → [C4F9CH2CH2OH·I]-
So far, this direct atmospheric sampling approach has been limited to laboratory measurements.
In contrast, the majority of atmospheric measurements of PFAS have relied on the collection of target analytes with offline quantitative analysis, such as LC-MS-MS for ionizable PFAS and GC-MS for neutral PFAS. Like many atmospheric pollutants, PFAS can be found in the gas phase, aerosol phase, or both.3 Aerosols can be collected for offline PFAS analysis through standard collection techniques such as filtration3 and impaction.8 However, analytical determination biases must be considered.
The most common method for the collection of gaseous PFAS is on a sorbent under flow (active sampling) or through diffusion (passive sampling). In either approach, the sorbent targeting PFAS is subject to competitive processes with other components of the atmospheric matrix that may be present at much higher levels.9 Typical sorbents are one or both of polyurethane foam (PUF) and polystyrene-divinylbenzene (XAD) porous resin. The sorbent(s) are used to separate PFAS from the atmosphere, extracted using solvent, concentrated through solvent evaporation, and then analyzed by GC-MS or LC-MS-MS. Air sampling methods with PUF and XAD are common for semi-volatile persistent organic pollutants (POPs) such as polychlorinated biphenyls. This was the basis for the first method to collect multiple PFAS from the atmosphere.10 In that active sampling method, large volumes (hundreds of m3) of the atmosphere containing gaseous PFAS were collected on PUF and XAD sorbents under high flow rates (several m3/hr). Many PFAS have much higher volatility than POPs, which means this method only collects relatively low-volatility PFAS efficiently. Method recoveries decreasing with increasing PFAS volatility have been reported,11 with losses of the more volatile PFAS in the analytical suite due to both the sampling and sample preparation steps.12 While losses can be quantified using internal standards,12 recoveries <50% for important PFAS, such as 4:2 and 6:2 fluorotelomer alcohols,13,14 limit the ability of these methods to detect PFAS at atmospherically relevant levels. Despite the drawbacks, this method is commonly used, especially in monitoring programs where PFAS is targeted opportunistically alongside other POPs15 because the equipment is widely available, standard procedures are in place, site personnel are trained, and the equipment is already deployed in the desired regions. Several other passive and active sampling methods based on PUF and XAD have subsequently been developed. Similar volatility-dependent losses have been reported for some of these active methods,3 indicating that these challenges with measuring volatile PFAS are common across active sampling methods designed with these sorbents. Since all active sampling methods are intended to be exhaustive, and passive sampling techniques are equilibrium-based, passive samplers based on PUF, XAD, or both will not be able to capture highly volatile PFAS effectively.16
Sampling of gaseous PFAS has, in some cases, undergone more selective targeting based on the unique physical properties of particular sub-classes of compounds. Some of the first measurements of gaseous acidic PFAS used carbonate-coated annular denuders.17 This is a standard method for selective collection of gas phase inorganic acids (such as nitric acid or hydrochloric acid) resulting from fossil fuel combustion.18 So long as the reactive capacity of the coating is not exceeded, the acidic analytes are accumulated quantitatively and irreversibly. This approach has been used in a few studies focused on ultra-short chain perfluorocarboxylic acids,19,20 with denuders typically extracted into aqueous solution for offline analysis. Thermal desorption (TD) sampling has also effectively targeted several classes of neutral PFAS.9,21,22 The TD technique uses highly porous materials (such as Tenax® and activated carbon) to exploit adsorptive surface interactions to trap analytes. Sorbent properties can be selected based on the volatility of the targets, including the use of sorbent mixtures to expand the analyte volatility range. The complex mixture inherent to atmospheric samples is then ideally fully extracted, which has been shown for some PFAS sub-classes. Still, high quality internal standards remain recommended to correct for or mitigate any method biases (such as GC inlet liner matrix effects).22 This is because the collected analytes are typically introduced to a GC directly upon being thermally displaced from the sorbent in a reversed gas flow, sometimes simultaneously subjected to cryotrapping to facilitate focused injection volumes. By quantitatively transferring all trapped molecules into the instrument, TD can achieve similar method detection limits to the more commonly used active sampling techniques described above in much smaller sampling volumes (<1 m3).14 Recent innovations in TD have expanded the suite of PFAS target analytes from atmospheric samples21 and are expected to continue to do so.
Ongoing challenges in measuring atmospheric PFAS
Determining whether PFAS are present as gases or in particles is critical for understanding their atmospheric fate and transport.23 This requires the separation of gaseous and particulate PFAS during sampling. Acidic PFAS, as terminal degradation products, can be subject to sampling artifacts during separations of these two atmospheric phases, particularly if the particles are sampled prior to the gases, for example, with a filter followed by a sorbent. Gaseous acidic PFAS can sorb to filter substrates such as quartz fiber, leading to a “blow on” artifact.24 Meanwhile, particulate acidic PFAS could repartition or be displaced by atmospheric gaseous acids (such as hydrochloric acid), leading to a “blow off” effect25 (Figure 1). As with other atmospheric acids, when sampling acidic PFAS, the gases should be collected prior to the particles, and characterization of the propensity of analytes to blow off of particles should be made in order to ascertain if additional collection media are required to trap liberated analytes following the filter.23
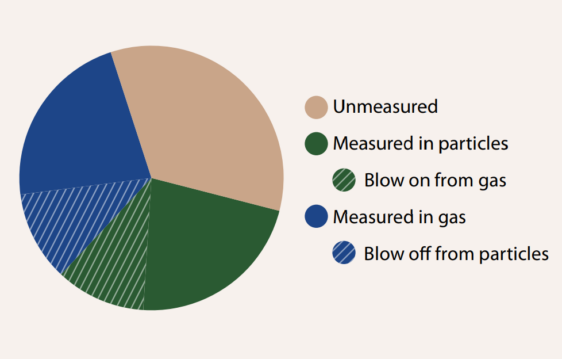
Lastly, the measurement of total organic fluorine (TOF) has increased our understanding of PFAS in the condensed phase.26 However, TOF methods developed to date require a condensed phase sample. Application of current TOF methods to the atmosphere requires a collection step to transfer gaseous and particulate analytes to the condensed phase. This is more challenging for the most volatile PFAS for which there are currently no robust atmospheric collection methods. Because there is selectivity inherent in any collection method that separates PFAS analytes from air, samples subjected to offline TOF analysis should be anticipated to be inherently incomplete (Figure 1). Thus, the current suite of separation methods developed for past PFAS compound sub-classes in the atmosphere cannot and should not be expected to be effective for use to give a true measure of TOF in the atmosphere. If in situ methods are developed, there is a much higher chance of obtaining such a measurement and capturing the atmospheric chemistry drivers that dictate the sources and fate of atmospheric PFAS.
Authors
Cora J. Young is an associate professor and the Guy Warwick Rogers Chair in Chemistry at York University in Toronto, Canada. She has been studying PFAS for over twenty years, focusing on their atmospheric measurement and fate.
www.cjygroup.com
Trevor C. VandenBoer is an assistant professor in the Department of Chemistry at York University. His expertise is the measurement of ultra-trace chemicals in the atmosphere, particularly atmospheric acids.
www.tcvandenboer.ca
References
(1) Young, C. J.; Mabury, S. A. Atmospheric Perfluorinated Acid Precursors: Chemistry, Occurrence and Impacts. Rev Environ Contam Toxicol 2010, 208, 1–110. https://doi.org/10.1007/978-1-4419-6880-7_1.
(2) Sunderland, E. M.; Hu, X. C.; Dassuncao, C.; Tokranov, A. K.; Wagner, C. C.; Allen, J. G. A Review of the Pathways of Human Exposure to Poly- and Perfluoroalkyl Substances (PFASs) and Present Understanding of Health Effects. Journal of Exposure Science and Environmental Epidemiology. Nature Publishing Group March 1, 2019, pp 131–147. https://doi.org/10.1038/s41370-018-0094-1.
(3) Ahrens, L.; Harner, T.; Shoeib, M.; Lane, D. A.; Murphy, J. G. Improved Characterization of Gas-Particle Partitioning for per- and Polyfluoroalkyl Substances in the Atmosphere Using Annular Diffusion Denuder Samplers. Environ Sci Technol 2012, 46 (13), 7199–7206. https://doi.org/10.1021/es300898s.
(4) Scott, B. F.; Spencer, C.; Mabury, S. A.; Muir, D. C. G. Poly and Perfluorinated Carboxylates in North American Precipitation. Environ Sci Technol 2006, 40 (23), 7167–7174.
(5) Feng, J.; Vet, R.; Cole, A.; Zhang, L.; Cheng, I.; O’Brien, J.; Macdonald, A. M. Inorganic Chemical Components in Precipitation in the Eastern U.S. and Eastern Canada during 1989–2016: Temporal and Regional Trends of Wet Concentration and Wet Deposition from the NADP and CAPMoN Measurements. Atmos Environ 2021, 254. https://doi.org/10.1016/j.atmosenv.2021.118367.
(6) Riedel, T. P.; Lang, J. R.; Strynar, M. J.; Lindstrom, A. B.; Offenberg, J. H. Gas-Phase Detection of Fluorotelomer Alcohols and Other Oxygenated per- and Poly Fluoroalkyl Substances by Chemical Ionization Mass Spectrometry. Environ Sci Technol Lett 2019, 6, 289–293. https://doi.org/10.1021/acs.estlett.9b00196.
(7) Bowers, B. B.; Thornton, J. A.; Sullivan, R. C. Evaluation of Iodide Chemical Ionization Mass Spectrometry for Gas and Aerosol-Phase per- and Polyfluoroalkyl Substances (PFAS) Analysis. Environ Sci Process Impacts 2022, 25 (2), 277–287. https://doi.org/10.1039/d2em00275b.
(8) Barton, C. A.; Butler, L. E.; Zarzecki, C. J.; Flaherty, J. M.; Kaiser, M. Characterizing Perfluorooctanoate in Ambient Air near the Fence Line of a Manufacturing Facility: Comparing Modeled and Monitored Values. J Air Waste Manage Assoc 2006, 56, 48–55.
(9) Roth, J.; Abusallout, I.; Hill, T.; Holton, C.; Thapa, U.; Hanigan, D. Release of Volatile Per-and Polyfluoroalkyl Substances from Aqueous Film-Forming Foam. Environ Sci Technol Lett 2020, 7 (3), 164–170. https://doi.org/10.1021/acs.estlett.0c00052.
(10) Martin, J. W.; Muir, D. C. G.; Moody, C. A.; Ellis, D. A.; Kwan, W.; Solomon, K. R.; Mabury, S. A. Collection of Airborne Fluorinated Organics and Analysis by Gas Chromatography/Chemical Ionization Mass Spectrometry. Anal Chem 2002, 74 (3), 584–590.
(11) Ahrens, L.; Shoeib, M.; Harner, T.; Lane, D. A.; Guo, R.; Reiner, E. J. Comparison of Annular Diffusion Denuder and High Volume Air Samplers for Measuring Per- and Polyfluoroalkyl Substances in the Atmosphere. Anal Chem 2011, 83, 9622–9628.
(12) Jahnke, A.; Ahrens, L.; Ebinghaus, R.; Berger, U.; Barber, J. L.; Temme, C. An Improved Method for the Analysis of Volatile Polyfluorinated Alkyl Substances in Environmental Air Samples. Anal Bioanal Chem 2007, 387 (3), 965–975. https://doi.org/10.1007/s00216-006-1008-y.
(13) Dreyer, A.; Matthias, V.; Temme, C.; Ebinghaus, R. Annual Time Series of Air Concentrations of Polyfluorinated Compounds. Environ Sci Technol 2009, 43, 4029–4036.
(14) Wu, Y.; Chang, V. W. C. Comparison of Solvent Extraction and Thermal Desorption Methods for Determination of Volatile Polyfluorinated Alkyl Substances in the Urban Atmosphere. Analytical Methods 2013, 5 (13), 3410–3417. https://doi.org/10.1039/c3ay40089a.
(15) Wong, F.; Hung, H.; Dryfhout-Clark, H.; Aas, W.; Bohlin-Nizzetto, P.; Breivik, K.; Mastromonaco, M. N.; Lundén, E. B.; Ólafsdóttir, K.; Sigurðsson, Á.; Vorkamp, K.; Bossi, R.; Skov, H.; Hakola, H.; Barresi, E.; Sverko, E.; Fellin, P.; Li, H.; Vlasenko, A.; Zapevalov, M.; Samsonov, D.; Wilson, S. Time Trends of Persistent Organic Pollutants (POPs) and Chemicals of Emerging Arctic Concern (CEAC) in Arctic Air from 25 Years of Monitoring. Science of the Total Environment 2021, 775. https://doi.org/10.1016/j.scitotenv.2021.145109.
(16) Shoeib, M.; Harner, T.; Sum, C. L.; Lane, D.; Zhu, J. Sorbent-Impregnated Polyurethane Foam Disk for Passive Air Sampling of Volatile Fluorinated Chemicals. Anal Chem 2008, 80 (3), 675–682. https://doi.org/10.1021/ac701830s.
(17) Martin, J. W.; Mabury, S. a; Wong, C. S.; Noventa, F.; Solomon, K. R.; Alaee, M.; Muir, D. C. G. Airborne Haloacetic Acids. Environ Sci Technol 2003, 37 (13), 2889–2897. https://doi.org/10.1021/es026345u.
(18) United States Environmental Protection Agency. Compendium of Methods for the Determination of Inorganic Compounds in Ambient Air: Determination of Reactive Acidic and Basic Gases and Strong Acidity of Atmospheric Fine Particles (<2.5 Μm) (Compendium Method IO-4.2); 1999.
(19) Zhang, B.; Zhai, Z.; Zhang, J. Distribution of Trifluoroacetic Acid in Gas and Particulate Phases in Beijing from 2013 to 2016. Science of the Total Environment 2018, 634, 471–477. https://doi.org/10.1016/j.scitotenv.2018.03.384.
(20) Ye, R. X.; Di Lorenzo, R. A.; Clouthier, J. T.; Young, C. J.; VandenBoer, T. C. A Rapid Derivatization for Quantitation of Perfluorinated Carboxylic Acids from Aqueous Matrices by Gas Chromatography-Mass Spectrometry. Anal Chem 2023, 95 (19), 7648–7655. https://doi.org/10.1021/acs.analchem.3c00593.
(21) Titaley, I. A.; De la Cruz, F. B.; Barlaz, M. A.; Field, J. A. Neutral Per- and Polyfluoroalkyl Substances in In Situ Landfill Gas by Thermal Desorption-Gas Chromatography-Mass Spectrometry. Environ Sci Technol Lett 2023, 10 (3), 214–221. https://doi.org/10.1021/acs.estlett.3c00037.
(22) Wu, Y.; Chang, V. W. C. Development of Analysis of Volatile Polyfluorinated Alkyl Substances in Indoor Air Using Thermal Desorption-Gas Chromatography-Mass Spectrometry. J Chromatogr A 2012, 1238, 114–120. https://doi.org/10.1016/j.chroma.2012.03.053.
(23) Tao, Y.; VandenBoer, T. C.; Ye, R. X.; Young, C. J. Exploring Controls on Perfluorocarboxylic Acid (PFCA) Gas-Particle Partitioning Using a Model with Observational Constraints. Environ Sci Process Impacts 2022, 25 (2), 264–276. https://doi.org/10.1039/d2em00261b.
(24) Arp, H. P. H.; Goss, K.-U. Irreversible Sorption of Trace Concentrations of Perfluorocarboxylic Acids to Fiber Filters Used for Air Sampling. Atmos Environ 2008, 42, 6869–6892.
(25) MacInnis, J. J.; VandenBoer, T. C.; Young, C. J. Development of a Gas Phase Source for Perfluoroalkyl Acids to Examine Atmospheric Sampling Methods. Analyst 2016, 141, 3765–3775. https://doi.org/10.1039/C6AN00313C. (26) Schultes, L.; Vestergren, R.; Volkova, K.; Westberg, E.; Jacobson, T.; Benskin, J. P. Per- and Polyfluoroalkyl Substances and Fluorine Mass Balance in Cosmetic Products from the Swedish Market: Implications for Environmental Emissions and Human Exposure. Environ Sci Process Impacts2018, 20 (12), 1680–1690. https://doi.org/10.1039/c8em00368h.
This article is featured in our October publication, 'PFAS: Unraveling the Analytical Challenges.' From cutting-edge analytical methods to the unexpected avenues of PFAS exposure in everyday items, explore the multifaceted challenges and solutions surrounding these pervasive contaminants.